A strongly emitting liquid-crystalline derivative of Y3N@C80: bright and long-lived near-IR luminescence from a charge transfer state
Delphine Felder-Flesch, informal collaboration with Prs. Paola CERONI and Francesco ZERBETTO, Dipartimento di Chimica Ciamician, Université de Bologne, Italy.
Metal-containing fullerenes, and particularly trimetallic nitride template endohedral metallofullerenes (TNT-EMFs), elicit increasing attention not only for their fascinating structure and the possibility to stabilize metallic nitrides, but also for their outstanding electronic and optical properties. TNT-EMFs are noteworthy electron-acceptors. They also possess larger absorption coefficients than C60 in the visible region, making them promising candidates for replacing the well-known phenyl-C61-butyric acid methyl ester (PCBM) in bulk heterojunction (BHJ) solar cells. They could then be used as potential auxiliary materials for singlet-exciton dissociation at the donor-acceptor interfaces, providing charge transport pathways across the semiconducting layer. Charge-carrier transport and light-emission efficiencies are crucially related to the molecular organization and degree of ordering, i.e. in thin film morphology (nanostructuration). It is widely recognized that self-organization by the formation of low-dimensional liquid-crystalline (LC) phases is a key strategy to control ordering and structuring of organic semiconductors as it helps to reduce or even suppress defect formation. Chemical functionalization of TNT-EMFs appears therefore as an original and promising approach to reach self-organization and to obtain processable materials. If a wide number of LC fullerenes have been studied since the early 90s, none study concerns the TNT-EMFs family.
Our main goal is to design and characterize TNT-EMF-based liquid crystals (1) and compare both their mesomorphic and photophysical behaviours with their related C60 counterpart (2) (Figure 1). In a first report [1] both fullerene entities (C60 and Y3N@C80) are chemically linked to two oligo(phenyleneethynylene) arms (OPE for short) mandating both self-organizing and absorbing properties. Experimentally, both the [5,6] and [6,6] types of double bonds can be potential reactive sites of TNT fullerenes, although the regioselectivity is usually very high.
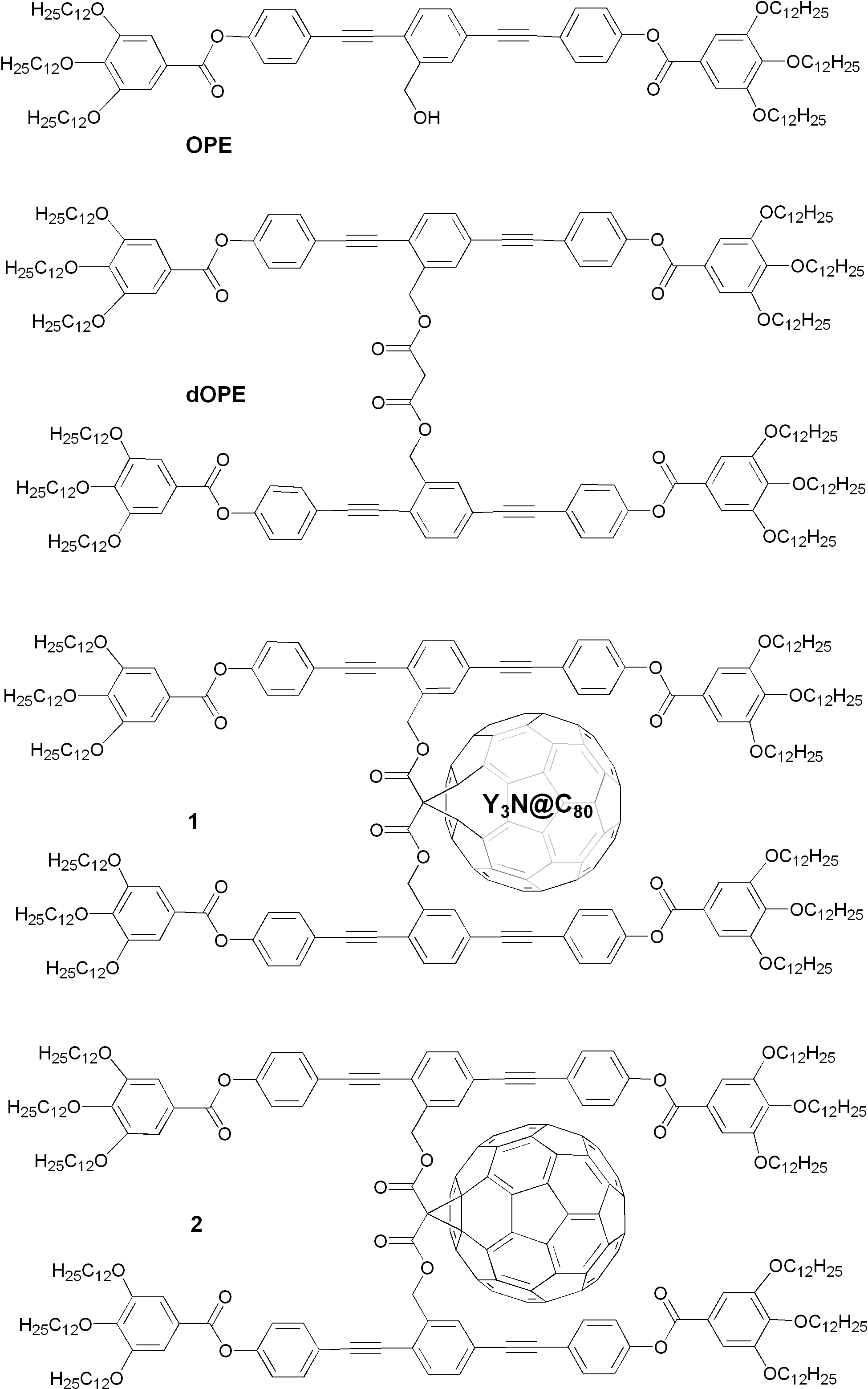
Figure 1 : Chemical structures of Y3N@C80> (1) and C60-based (2) dyads and their common malonate intermediate dOPE.
NMR is a powerful technique to distinguish between these two addition patterns, but the low solubility of most of the TNT-EMF derivatives usually prevents their NMR characterization and the use of 13C labelled samples or solvent mixtures with CS2 are required. The presence of twelve long alkyl chains however made possible the complete NMR characterization of the Y3N@C80 derivative 1 in pure chloroform and confirmed that the product is a [6,6]-bridged fulleroid.
Photophysical investigations were performed in the laboratory of Prof. Paola Ceroni, U Bologna. The absorption spectra of 1 and 2 in toluene solution show the contribution of the two constituent chromophores: the band at 326 nm is mainly due to the OPE units, while the absorption at λ>380 nm is characteristic of the fullerene core (Figure 2a). It is worth noting that the molar absorption coefficient of 1 in the visible region is much higher than that of 2 and extends up to 750 nm because of the endohedral fullerene core.
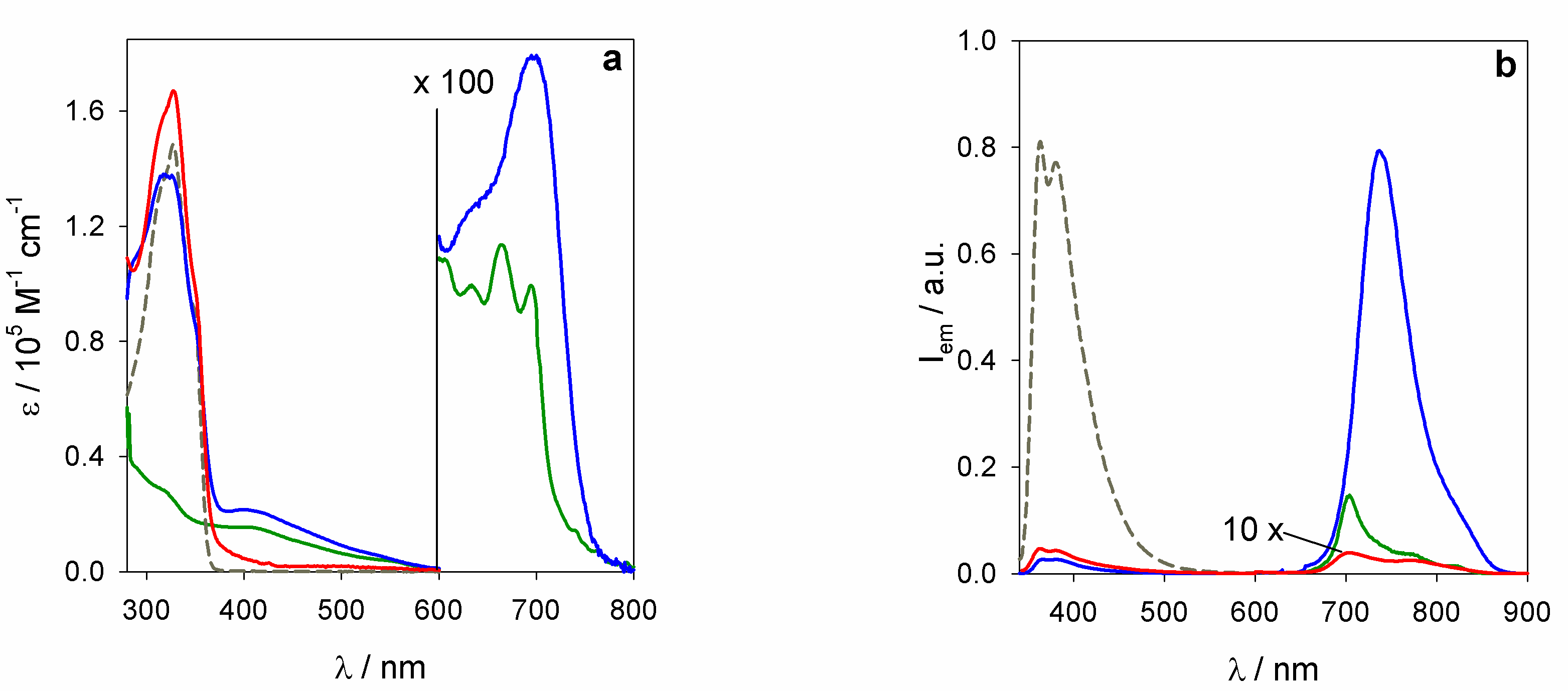
Figure 2 : Absorption (a) and emission (b) spectra of 1 (blue line), 2 (red line), dOPE (dashed gray line), and Y3N@C80 (green line) in de-aerated toluene solution at 298 K. λex= 325 nm. The emission intensities are registered for isoabsorbing solutions at the λex.
The emission spectra of 1 and 2 in de-aerated toluene solutions (Figure 2b) show two bands: the first one at ca. 365 nm, which is centered on the OPE moiety and is strongly quenched (>20 times) compared to model compound dOPE, and the second one in the 680-900 nm region, which can be attributed to the fullerene core (Table 1). The quenching of the OPE fluorescence can be attributed to a 100% efficient energy transfer. Indeed, the same fullerene emission intensity was recorded upon excitation of two iso-absorbing solutions of 1 at 320 nm, where most of the light is absorbed by the pending OPEs, and at 405 nm, where only the fullerene absorbs light (Figure 1). The same result was observed for compound 2. Therefore, the two OPE units act as extremely efficient light-harvesting antennae for the sensitization of the fullerene emission.
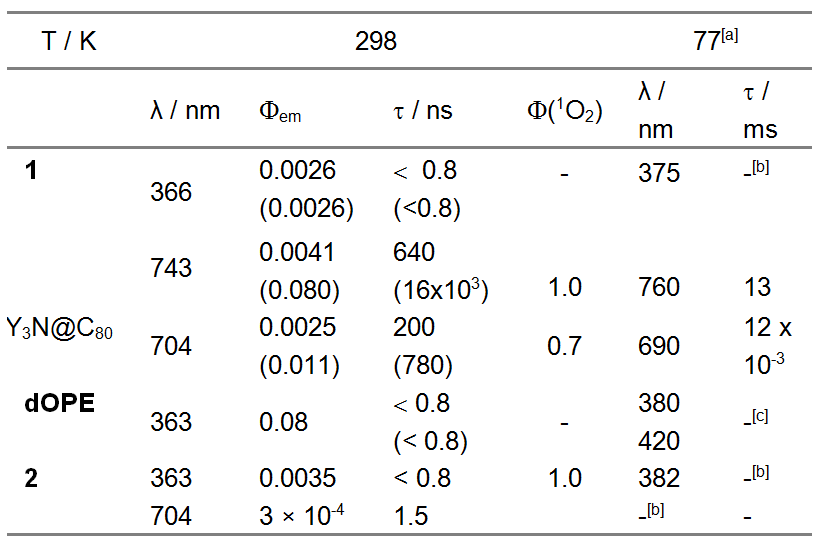
Table 1 : Emission properties of 1, 2, dOPE and Y3N@C80 in air-equilibrated or deaerated (values in brackets) toluene solution, unless otherwise noted. [a] In toluene:ethanol 1:1 (v/v) rigid matrix. [b] The emission intensity is too low.
The most striking difference between the two derivatives concerns the fullerene core emission: in the case of 2, a very weak fluorescence (Φem = 3 x 10-4) with a lifetime of 1.5 ns is observed, as expected for C60 derivatives. In contrast, compound 1 exhibits outstanding luminescence properties in the near-IR region, even larger than the non-functionalized model Y3N@C80. As reported in Table 1, the emitting excited state of 1 is: (i) slightly lower in energy compared to the non-functionalized endohedral fullerene, (ii) quite highly emitting (0.08 in de-aerated solution), (iii) extremely long-lived (16 μs at 298 K, 20 times higher than Y3N@C80 and 13 ms at 77 K), and (iv) highly sensitive to the presence of dioxygen in fluid solution (vide infra). The same emission band is observed in the solid phase, both in the amorphous and LC mesophase, with a strong quenching by dioxygen.
Dioxygen quenches efficiently the emission of 1 also in solution with a rate constant kq = 8 x 108 M-1 s-1. This value is slightly lower than that of the pristine Y3N@C80 (kq = 2 x 109 M-1 s-1), consistent with an embedding of the fullerene core by the OPE units of 1. Quenching by dioxygen (Figure 3) leads to sensitization of 1O2 emission at 1270 nm with a quantum yield of 1.0 and 0.7 for 1 and Y3N@C80, respectively (Table 1). The fluorescence of compound 2 is not quenched by dioxygen because of the very short lifetime, which prevents dynamic quenching. However, 2 can sensitize 1O2 by its lowest lying triplet excited state (ESI) with efficiency close to 1, as expected for C60 derivatives.
Quenching of the luminescence of 1 has been observed upon addition of ferrocene with kq = 6 x 109 M-1 s-1, a value lower than that of Y3N@C80 (kq = 1 x 1010 M-1 s-1) because of the embedding effect of the OPE units of 1. The quenching occurs by photoinduced electron-transfer from ferrocene to 1 since the ferrocene is easy to reduce and not to oxidize and it has no excited state lower than that of 1.
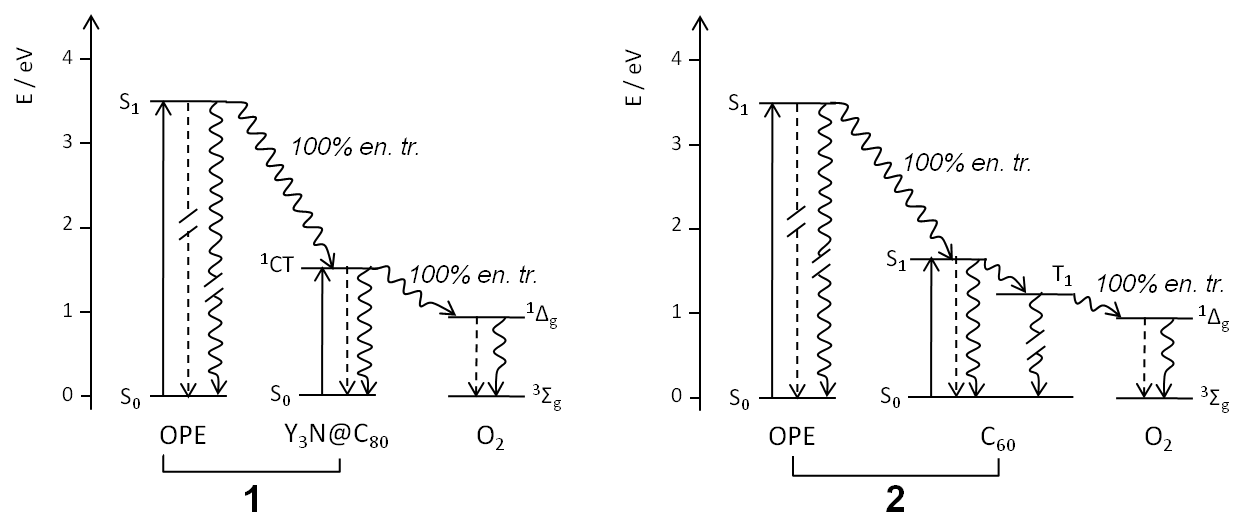
Figure 3 : Energy level diagrams showing the most relevant radiative (straight lines) and non-radiative (wavy lines) processes for 1. The excited states not relevant to the present discussion have been omitted for clarity reasons.
DFT calculations were performed in collaboration with Prof. Francesco Zerbetto, U Bologna. To further explore the nature of the long-lived emitting excited state, density functional theory (DFT) calculations were performed (Figure 3) on Y3N@C80 and compared to those obtained for Sc3N@C80, a closely related EMF with similar absorption spectrum, which does not emit.
The DFT optimized geometries at B3LYP/6-311G*/ECP level agreed with the structures proposed earlier. Both Sc3N and Y3N are planar. The amount of intramolecular charge transfer is larger for Y3N@C80 than Sc3N@C80, with the Y3N doped-cage that receives 3.84(4.99) electrons while the corresponding value for the Sc3N doped-cage is 2.98(3.41) with the B3LYP(HSE06) functional. The large values of charge-transfer suggest that molecule-cage Coulomb interactions play an important role in the structure and properties of these endohedral clusters. Further time-dependent DFT and ZINDO/S calculations showed that the lowest electronically excited singlet state S1 of both Sc3N@C80 and Y3N@C80 is mainly a HOMO-LUMO transition. The HSE06/6-311G*, CAM-B3LYP/6-311G* and ZINDO/S results locate S1 of Y3N@C80 at 2.02, 2.55, and 1.72 eV. For Sc3N@C80, the corresponding values are 1.72, 2.20, 1.74 eV. A crucial difference between Sc3N@C80 and Y3N@C80 emerges when the molecular orbitals involved in S1 are inspected visually. Figure 4 shows the HOMO’s and LUMO’s of the two endohedral clusters. In the non-emitting, short-lived S1 state of Sc3N@C80 the electron excitation is spread over the entire molecule: both the cage and the endohedrally confined Sc3N participate in the excitation.
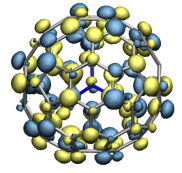
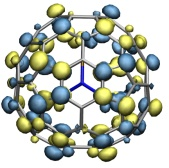
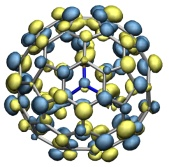
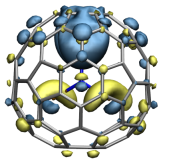
Figure 4 : HOMO of Sc3N@C80, b) HOMO of Y3N@C80, c) LUMO of Sc3N@C80, d) LUMO of Y3N@C80.
In the emitting, long-lived S1 state of Y3N@C80 the electron excitation has a strong charge transfer character from the carbon cage to the endohedrally trapped Y3N. The peculiar photophysical differences between Sc3N@C80 and Y3N@C80 are now easily accounted for. The electron-excitation to the cage-screened Y3N determines the long lifetime of S1 and inhibits the typical fullerenic de-activation pathway characterized by very low emission quantum yields.
Liquid-crystalline properties were investigated at IPCMS by Drs. B. Donnio and B. Heinrich. Fullerene adducts 1 and 2 are amorphous in the pristine state, but they self-organize into a LC mesophase during the first heating (at ca. 70°C for 1 and ca. 80°C for 2).The mesophase is retained upon cooling down to room-temperature, and also recovered on cooling after a further heating beyond the isotropization temperature, at approximately 80°C for 1 and 100°C for 2.
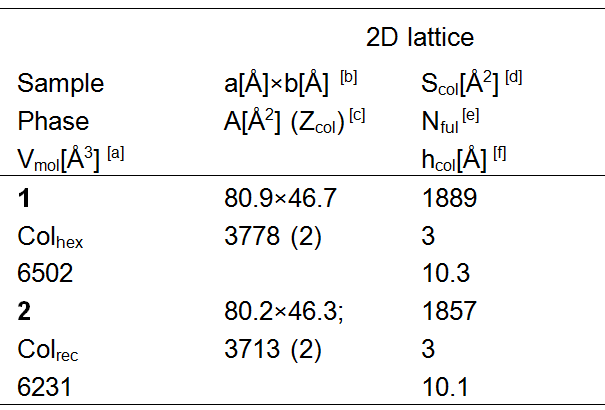
table 2 : Geometrical parameters of mesophases obtained from XRD at 20°C. [a] Calculated molecular volume.[b] Lattice parameters of the columnar phase.
The complete elucidation of the self-organizing behaviour was achieved by SAXS. Columnar mesophases were readily recognized from diffraction patterns, which are composed of the usual diffuse scattering at 4.5 Å indicative of the liquid-like lateral packing of the aliphatic chains and mesogenic OPE cores, and of up to 4 sharp reflections with the spacing ratio 1:3:2:7 in the small-angle region indicative of a 2D-lattice with hexagonal geometry (the reflection at 7 was not detected for 1). As no further small-angle reflection is visible in the patterns of 1, the mesophase is assigned to Colhex. One additional, weak signal located at twice the spacing of the first reflection of the series is detected in patterns of 2 and the real lattice therefore includes several hexagonal cells, presumably under the effect of small shifts in orientation and/or position between neighbouring columns, induced by its more symmetrical molecular structure (with respect to that of 1). The doubling of the hexagonal sub-lattice then generates a primitive rectangular lattice containing two columns, assigned to a Colrec phase with pseudo-hexagonal geometry.
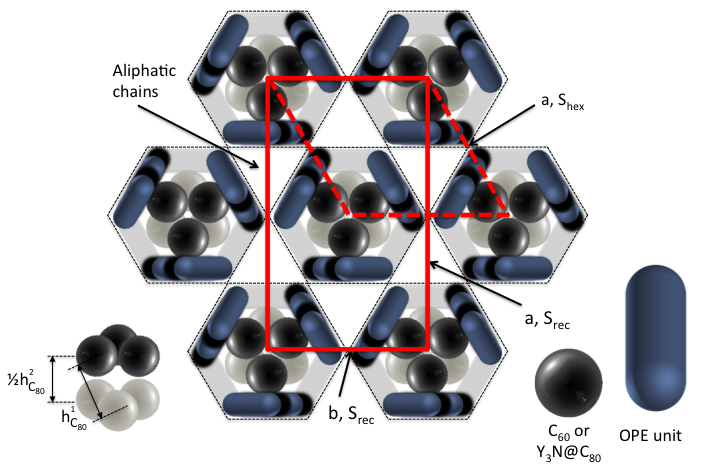
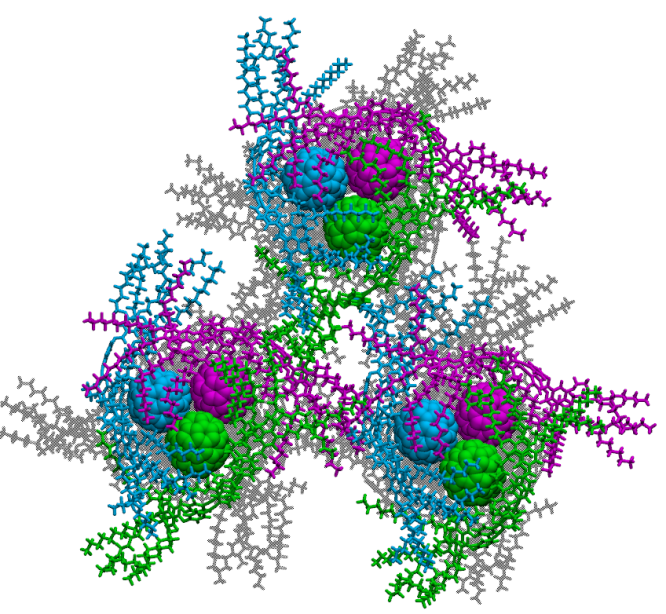
Figure 5 : (Top) Schematic view of the supramolecular packing of adducts 1 and 2 (solid and dotted red lines: Shex and Srec lattices of the Colhex and Colrec phases, respectively; dotted-black lines: Kagome lattice). (Bottom) Computer modelling of a supercell of 9 molecules of 1 arranged as in (a), the different colours are used to assist the eye.
Except for the lattice doubling, both columnar structures appear overall very similar. The grafting of C60 or TNT-EMF C80 unit onto the central bridge of dOPE generates an unusual triblock architecture which leads to the triple micro-segregation between fullerenes, mesogenic cores and chains in different zones separated by interfaces constraining the organization similarly to what is found with the complex “multi-color tiling” mesophases generated by polyphilic systems. A further similitude between both organizations consists in the size of columns: their similar cross-sections Scol (about 1.5% larger in 1 and in both cases nearly independent of temperature) lead to slice thicknesses hmol = Vmol/Scol comprised of between 3.4 and 3.6 Å. These values represent about one third of the distance between first neighbour’s fullerenes obtained from crystalline phases (about 10 and 11 Å, for C60 and C80, respectively) and column cross-sections contain therefore in the average Nful =3 (Table 2). This suggests the image of successive molecular plates with a core of 3 interacting fullerenes stacked into columns with an alternating 60° rotation, yielding a strand of hexagonally close packed bowls, as schematized in Figure 5. The associated three pairs of OPE mesogens are pressed again the fullerene strands and due to the short spacers are spatially constrained and form a sort of separating two-dimensional net between the fullerenes strands and the aliphatic chains tubes, reminiscent to aKagome lattice (Figure 4), i.e. a periodic 2D tiling of regular hexagons (filled by hcp stacked fullerenes) and triangles (filled by the molten aliphatic chains) in a 1:2 ratio (in relatively good agreement with the respective volume fractions). The lateral mesogens may adopt various orientations around a preferential one that lies between the parallel and perpendicular limit cases, but likely closer to the perpendicular orientation.
Structural calculations. Optimization of the structure of a super cell made by 9 molecules of 1 using a protocol employed before yielded a cell of 78.8 x 45.5 Å (Figure 3b) that compares well with the XRD data of 80.9×46.7 Å. The calculations also provide the value of hcol (ca. 11 Å) in agreement with XRD data.
In conclusion, we have synthesized the first example of a liquid-crystalline derivative of Y3N@C80 which shows good solubility and remarkable photophysical properties: OPE units acts as 100% efficient light-harvesting antennae to sensitize a bright and long-lived fullerene core emission. These luminescence properties are retained in the mesophase and, coupled to a quite strong absorption in the visible region extending up to 750 nm, open up a variety of applications, ranging from photovoltaics to near-IR luminescent sensors with time-gated detection to shut down fluorescence of the matrix or competing species. The grafting of the mesomorphic dOPE onto the fullerenes promotes mesomorphism in the fullerene adducts, with the induction of columnar phases resulting from the triple segregation between the fullerene (core), mesogens (walls) and chains (continuous medium) accordingly to a Kagome lattice; this particular multi-color tiling is driven by the simultaneous tendency of the fullerenes to aggregate face-to-face and to strongly segregate from the organic pending groups.